Introduction:
Thermoelectricity deals with the conversion of waste heat into electricity by exploiting the inherent coupling between thermal and electrical properties. It becomes attractive predominantly in the recent perspective that unprecedented need for energy clashes with the necessity of reducing carbon emission owing to the environmental issues.
Decreasing of fossil fuel enforces the mankind to think about sustainable supply of energy to the world’s population. Thermoelectric (TE) phenomena, involving the conversion between thermal and electrical energy, and providing a method for heating and cooling materials, are expected to play an important role in meeting the energy challenge of the future. In addition, TE materials have potential application in the field of solid state cooling viz., peltier cooler. Unfortunately, the application of TE devices is very limited due to its low conversion efficiency. As of now TE devices have niche applications for space missions, laboratory equipment and medical applications, where energy availability, reliability, predictability and silent operation of the modules are more important than its cost and energy efficiency.
There exist various thermoelectric materials for different use purpose. Half-Heusler (maximum ZT ~ 1.0 for both n- and p-type at 500–8000C) is environmentally friendly, mechanically and thermally robust though cost may be an issue if a lot of Hf is needed. Therefore, they have attracted intensive research interest.
Structure and physical properties of Half-Heusler materials:
Half-Heuslers consists of XYZ as the main chemical composition, where X can be a transition metal, a noble metal, or a rare-earth element, Y is a transition metal or a noble metal, and Z is a
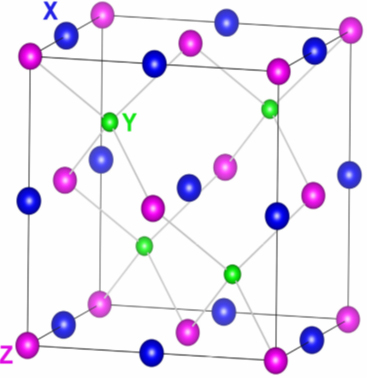
Fig 1: Crystal structure of a half-Heusler. The blue, green and pink dots correspond to X, Y, and Z atoms in half-Heuslers
main group element. As shown in Fig.1, XYZ forms a MgAgAs type of structure (space group F-43m), where X, Y, and Z atoms form three interpenetrating face-centered-cubic sublattices by occupying Wyckoff positions 4b (1/2, 1/2, 1/2), every other of 4c (1/4, 1/4, 1/4), and 4a (0, 0, 0) positions, respectively. The remaining (1/ 4, 1/4, 1/4) positions are empty. With such an atomic configuration, the strong hybridization of d states of the X and Y atoms induces a band gap in half-Heuslers. Based on calculation, the gap values vary from about 0.1 eV to 3.7 eV based on different compositions, the valence electron count (VEC) per unit cell and the average atomic number. There are theoretical calculations which show that half-Heuslers with 18 VEC per unit cell are stable and have a band gap in the range of 0–1.1 eV, which is suitable for moderate temperature thermoelectric applications. However, high thermal conductivity (6.7–20Wm-1 K-1 at room temperature) of half-Heusler is a major disadvantage for thermoelectric applications.
Thermoelectric Figure of Merit – Optimization
Being a solid state device with no moving parts; thermoelectric (TE) devices are silent, reliable, durable and scalable. The devices use just two type of legs i.e. n and p-type leg in series. Both the refrigeration and power generation may be accomplished using the same module. It is noteworthy to mention that NASA has used this principal to provide hundred watts of electrical power for deep space probes such as Voyager I and II and the Cassini mission to Saturn. However, commercial application of the device is limited by the efficiency of the device. The performance of a TE material is quantified in figure of merit, ZT=σS2T/κ; the term S2σ is called power factor (PF), where S, σ and κ are thermopower, electrical conductivity and thermal conductivity, respectively. Recent days, the best TE materials available have ZT ≈ 0.9. It is acceptable only for niche application, but economically competitive and commercial viable thermoelectric refrigerator require ZT ≈3. ZT can be enhanced either by maximize the PF through optimal doping and band engineering or reduce the lattice κ by nanostructuring through phonon engineering. Strategies to accomplish this goal have focused on increasing the average energy per carrier through energy filtering, carrier localization in narrow bands in quantum confined structures or the introduction of resonant levels. At the same time, as mentioned above, electrical conductivity, s (=1/r) must be optimized by properly adjusting the concentration of charge carriers (n) and maximizing their mobility. M. S. Dresselhaus et. al. also advocated for low dimensional materials as next generation TE materials, where two ideas are dominant. Firstly, the introduction of nanoscale constituents would introduce quantum confinement effects to enhance the PF. Secondly, the many internal interfaces found in nanostructures would be designed so that k would be reduced more than the s, based on differences in their respective scattering length. Experiments confirmed that the scattering of long and mid wavelength phonons can substantially enhance ZT by employing a high density of nanometer-sized grain boundaries. From this viewpoint, there are two representative methods of controlling and enhancing the performance of a TE material: nanostructuring as an intrinsic method and composite as an extrinsic method.
Why Half Hausler?
Half-Heuslar alloys (HH) with valence electron count 18, are very attractive to exploit as potential mid-temperature TE materials due to their narrow band gap and sharp slope of the density of states near the Fermi level. HH compounds, MNiSn (M=Ti, Zr or Hf) have become important TE material for converting heat into electricity in the temperature range 5000C to 8000C. Because of their high thermoelectric performance, low toxicity, relatively inexpensive elemental composition, robust thermal and mechanical properties, these materials exhibit semiconducting transport properties although they are ‘intermetallic’ compounds. Hence, it may be considered as rigid band semiconductor within Zintal compounds framework. It is noteworthy to mention that recently ZT ~1.5 has been obtained at 700K for n-type Mg3(SbBi)2 Zintal compound. However, the substitution of atoms can effectively decrease the lattice thermal conductivity via point defect phonon scattering and may tune the band structure, thus electrical properties of the material. Further, phonon scattering at the grain boundary due to presence of nano particle decreases the thermal conductivity in HH alloys. The recent approach to enhance the efficiency of the Hausler alloy is to synthesis a mixed Hausler and half-Heusler alloy with coherent phase boundary.
Resurgence of Research on Half Hausler alloys as a Thermoelectric material
Recently half-Heusler alloy are in the centre of the focus for the mid temperature waste heat management. Half-Heusler alloy draws the attention of researcher as potential candidate of TE material in the temperature range 300-7000C. The impediment to achieve high ZT in MNiSn ( Zr, Hf, Ti) half-Hausler alloy is high thermal conductivity. Reduction of thermal conductivity is achieved by incorporation of nano-phase in the matrix of MNiSn alloy. Power-factor might be modified by doping at the Sn-site and thermal conductivity by isoelectronic substitution of Zr on the Hf-site of the MNiSn (M=Zr, Hf, Ti). Theoretically it can be shown that mass disorder by alloying helps to reduce the thermal conductivity. It is noteworthy to mention that MCoSb is p-type thermoelectric material and counterpart of MNiSn for device fabrication. MCoSb composite having half-Heusler matrix with different size and CoSb impurity phase enhances ZT. High ZT of MCoSb half-Heusler alloy is also achieved by nano-composite approach using ball milling and hot pressing. Introduction of metallic phase nano-inclusion and full Heusler in the half-Heusler matrix is the new topic and worth further exploration to achieve high ZT via band structure engineering, scattering and energy filtering.
References:
- J. Snyder et.al. Nat. Mater. 7, 105 (2008).
- Ahn, K. Biswas, J. He, I. Chung, V. Dravid and M. G. Kanatzidis, Energy Environ. Sci. 6, 1529 (2013).
- Chen and Z. Ren, Materials Today 16, 387 (2013).
- Ahmad, K. Hoang, and S. D. Mahanti, Phys. Rev. Lett. 96, 056403 (2006).
- -S. Kim, N. A. Heinz, Z. M. Gibbs, Y. Tang, S. D. Kang, and G. J. Snyder, Mater. Today 20, 452 (2017).
- Yan, G. Joshi, W. Liu, Y. Lan, H. Wang, S. Lee, J. W. Simonson, S. J. Poon, T. M. Tritt, G. Chen, and Z. F. Ren, Nano Lett. 11, 556 (2011).